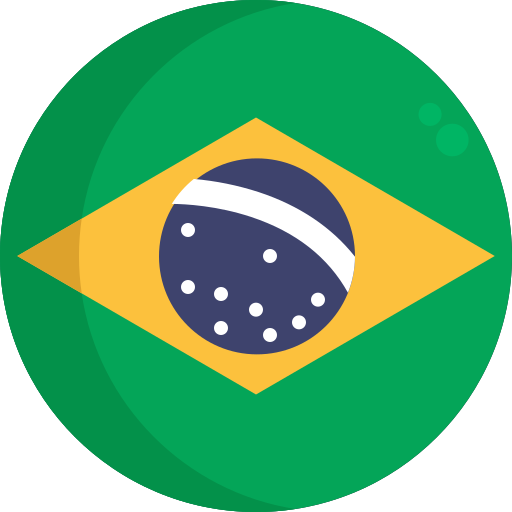
EVOLUTION OF SCAPULA SIZE AND SHAPE IN DIDELPHID MARSUPIALS (DIDELPHIMORPHIA: DIDELPHIDAE)
2009; Oxford University Press; Volume: 63; Issue: 9 Linguagem: Inglês
10.1111/j.1558-5646.2009.00720.x
ISSN1558-5646
Autores Tópico(s)Morphological variations and asymmetry
ResumoEvolutionVolume 63, Issue 9 p. 2438-2456 Free Access EVOLUTION OF SCAPULA SIZE AND SHAPE IN DIDELPHID MARSUPIALS (DIDELPHIMORPHIA: DIDELPHIDAE) Diego Astúa, Diego Astúa Laboratório de Mastozoologia, Departamento de Zoologia, Centro de Ciências Biológicas, Universidade Federal de Pernambuco. Recife, PE, Brazil E-mail: diegoastua@ufpe.brSearch for more papers by this author Diego Astúa, Diego Astúa Laboratório de Mastozoologia, Departamento de Zoologia, Centro de Ciências Biológicas, Universidade Federal de Pernambuco. Recife, PE, Brazil E-mail: diegoastua@ufpe.brSearch for more papers by this author First published: 26 August 2009 https://doi.org/10.1111/j.1558-5646.2009.00720.xCitations: 39AboutSectionsPDF ToolsRequest permissionExport citationAdd to favoritesTrack citation ShareShare Give accessShare full text accessShare full-text accessPlease review our Terms and Conditions of Use and check box below to share full-text version of article.I have read and accept the Wiley Online Library Terms and Conditions of UseShareable LinkUse the link below to share a full-text version of this article with your friends and colleagues. Learn more.Copy URL Share a linkShare onFacebookTwitterLinked InRedditWechat Abstract The New World family Didelphidae, the basal lineage within marsupials, is commonly viewed as morphologically conservative, yet includes aquatic, terrestrial, scansorial, and arboreal species. Here, I quantitatively estimated the existing variability in size and shape of the Didelphidae scapula (1076 specimens from 56 species) using geometric morphometrics, and compared size and shape differences to evolutionary and ecologic distances. I found considerable variation in the scapula morphology, most of it related to size differences between species. This results in morphologic divergence between different locomotor habits in larger species (resulting from increased mechanical loads), but most smaller species present similarly shaped scapulae. The only exceptions are the water opossum and the short-tailed opossums, and the functional explanations for these differences remain unclear. Scapula size and shape were mapped onto a molecular phylogeny for 32 selected taxa and ancestral size and shapes were reconstructed using squared-changed parsimony. Results indicate that the Didelphidae evolved from a medium- to small-sized ancestor with a generalized scapula, slightly more similar to arboreal ones, but strikingly different from big-bodied present arboreal species, suggesting that the ancestral Didelphidae was a small scansorial animal with no particular adaptations for arboreal or terrestrial habits, and these specializations evolved only in larger-bodied clades. The shoulder girdle of therian mammals is morphologically simpler than that of basal mammals (monotremes) or reptiles, being constituted of fewer elements (Jenkins and Weijs 1979). The typical therian scapula bears a lateral surface divided in two fossae by a scapular spine, which constitutes an important therian diagnostic character (Sánchez-Villagra and Maier 2002). This scapula, in spite of its apparent structural simplicity, has been considered a complex morphological structure, with different ossification centers and a shape simultaneously influenced by mechanical requirements and developmental and phylogenetic constraints (Monteiro and Abe 1999). Scapulae are particularly suited for ecomorphology and morphological evolution studies, because they can be easily morphometrically quantified and yet contain considerable variation related to ecology and phylogeny. Variation in scapula size and shape has been studied in several eutherian mammal groups, such as rodents (Leamy and Atchley 1984; Swiderski 1993; Taylor and Siegel 1995), xenarthrans (Monteiro and Abe 1999), primates (Taylor 1997), and cetaceans (Smith et al. 1994). The three main lineages of living mammals (monotremes, marsupials, and eutherians) are most clearly characterized by the distinctiveness in their reproductive modes. In marsupials, young are born at a very altricial state and have to complete their development attached to the teats (Tyndale-Biscoe and Renfree 1986). The pups, born after a gestation of approximately 13–35 days with a completely cartilaginous skeleton (Oliveira et al. 1998), have to reach the teats by themselves. They are thus born with their forelimbs precociously developed with digits and claws, capable of grasping and pulling the body, whereas the hindlimbs are simple buds or pads and several organs or systems are still unformed (Tyndale-Biscoe and Renfree 1986; Martin and Mackay 2003). Along with these precocious forelimbs, neonate marsupials also present a transient cartilaginous shoulder arch, consisting of several elements that later disappear and resemble the primitive condition found in monotremes (Klima 1987; Sears 2004). The development and ossification of the forelimbs and shoulder girdle prior to those of the hindlimbs appear to constitute a marsupial synapomorphy (Sánchez-Villagra 2002; Weisbecker et al. 2008). This early investment in the development of functional forelimbs apparently leads to developmental constraints in their morphological variation, thus resulting in a lower morphological diversity in their scapula when compared to their pelves (Sears 2004). These constraints are believed by some authors (e.g., Lillegraven 1975) to have limited their morphological diversification, reflected, for example, in the fact that marsupial scapulae are also less diverse than eutherian scapulae (Sears 2004). Among marsupials, members of the New World order Didelphimorphia constitute the most basal lineage (Amrine-Madsen et al. 2003; Cardillo et al. 2004), and are often considered to be morphologically conservative. Living Didelphimorphia have been used as models for ancestral, unspecialized mammals, particularly the big-bodied opossums of the genus Didelphis (e.g., Jenkins 1971; Jenkins and Weijs 1979). The Didelphimorphia are currently constituted by a single family, Didelphidae, with 19 genera and more than 90 species (Gardner 2005). In spite of being considered conservative, didelphids include a wide range of habitat use or locomotion modes, with exclusively terrestrial (Monodelphis, Metachirus) or arboreal (Caluromys, Micoureus) forms and the sole semiaquatic known marsupial, Chironectes minimus (Vieira 2005a,b). This variety of locomotor habits, coupled with a wide range in body sizes, varying from 10 g (Monodelphis, Hyladelphys) to more than 2000 g (Didelphis), allows them to occupy all the available strata in both forested and open habitats. When compared to most marsupials, Didelphidae are considered to present an intermediate state of development at birth (between highly precocial and altricial species), a shoulder arch with articular surfaces at birth (postulated to be the marsupial ancestral condition), and require an extensive amount of crawling from the urogenital sinus to the teats (Gemmell et al. 2002; Sánchez-Villagra and Maier 2003; Sears 2004). Didelphid marsupials therefore constitute a particularly interesting group to investigate the effects of size and ecology in scapula morphology in a primitive monophyletic group that shares the same ontogenetic constraints to morphological diversification, and yet constitute a considerable ecological radiation. In this study, I evaluated the morphometric variation and differentiation (i.e., in size and shape) of the scapulae of living species of Didelphimorphia and assessed the relation between scapula size and shape and of these variables to the species’ ecology (habitat use or locomotion) and phylogenetic history. Finally, I used these data to reconstruct the Didelphidae ancestral scapular size and shape, and thus to infer the evolution of size and shape of this structure and consequently discuss on the validity of using some of these taxa as models for generalized primitive mammals. Material and Methods SAMPLE I examined all suitable specimens with postcranial elements available from the following mammal collections: American Museum of Natural History (AMNH), Field Museum of Natural History (FMNH), Natural History Museum, University of Kansas (KU), Museu Nacional do Rio de Janeiro (MN), Museum of Southwestern Biology (MSB), Museo de Historia Natural de la Universidad Nacional Mayor de San Marcos (MUSM), Museum of Vertebrate Zoology (MVZ), Museu de Zoologia da Universidade de São Paulo (MZUSP), Sam Noble Oklahoma Museum of Natural History (OMNH), Mammal Collection, Departamento de Zoologia da Universidade Federal de Minas Gerais (UFMG), National Museum of Natural History (USNM), University of Wisconsin Zoological Museum (UWZM). These were complemented with additional material for particular taxa from the Bell Museum of Natural History, University of Minnesota (MMNH), Museum of Natural Science, Louisiana State University (LSUMZ), Coleção de Mamíferos, Universidade Federal de Santa Catarina (UFSC), and additional material kindly made available by Drs. Rui Cerqueira (URJ), Lena Geise (UERJ), Robert S. Voss (AMNH), and David Flores (PIDBA-UNT). I examined a total of 1079 specimens, from 16 genera and 56 species of Didelphidae. A complete listing of species included in this study, with their respective sample sizes, is presented in Table 1. Samples were limited to approximately 30 males and 30 females to maximize taxonomic representativeness, for those few taxa well represented in the examined collections. For rarer taxa all available specimens were examined. Specific criteria used for selecting samples for each species are detailed in Astúa de Moraes (2004), and can be obtained upon request, as well as a complete list of the specimens examined. Table 1. Specimens examined and respective sample sizes. Asterisks indicate species that were also used in the ancestral size and shape reconstruction. Genus/species n Genus/species N Caluromys Marmosops (cont.) C. derbianus 23 M. parvidens* 5 C. lanatus* 13 M. paulensis 15 C. philander* 19 Metachirus Caluromysiops M. nudicaudatus* 59 C. irrupta* 6 Micoureus Chironectes M. constantiae 14 C. minimus* 23 M. demerarae* 59 Didelphis M. paraguayanus* 27 D.albiventris* 64 M. phaeus 2 D. aurita 37 M. regina* 25 D. marsupialis* 42 Monodelphis D. pernigra 6 M. adusta* 5 D. virginiana* 58 M. americana 3 Glironia M. brevicaudata* 3 G. venusta* 1 M. dimidiata 1 Gracilinanus M. domestica* 40 G. agilis* 63 M. glirina 4 G. dryas 2 M. kunsi 1 G. marica 2 M. palliolata 7 G. microtarsus* 15 Philander Lestodelphys P. andersoni 3 L. halli* 1 P. frenatus* 21 Lutreolina P. mcilhennyi* 5 L. crassicaudata* 23 P. opossum* 71 Marmosa Thylamys M. mexicana* 14 T. cinderella 1 M. murina* 39 T. elegans 8 M. robinsoni* 37 T. karimii 9 Marmosops T. macrurus 4 M. bishopi 1 T. pallidior* 27 M. fuscatus 5 T. pusillus 6 M. impavidus* 8 T. sponsorius 15 M. incanus* 64 T. venustus* 1 M. invictus 8 Tlacuatzin M. noctivagus* 42 T. canescens* 2 M. ocellatus 16 Total 1079 I used only adult specimens to avoid influence of ontogenetic variation. Specimens were considered adults when presenting fully erupted upper and lower third premolars and fourth molars (Tyndale-Biscoe and Mackenzie 1976; Tribe 1990; Luckett and Hong 2000). I followed here the taxonomic arrangement proposed by Gardner (2005). Didelphid genera not included in the latter arrangement such as Cryptonanus and Chacodelphys (Voss et al. 2004; Voss et al. 2005) were not included here. IMAGES AND LANDMARKS I took digital images of the right scapula in lateral view with a Nikon Coolpix 995 (Nikon Americas Inc, Melville, NY) camera at a resolution of 1280 × 960 pixels, and all the images included a ruler for scale. For those specimens in which the right scapula was damaged or missing, the left scapula was photographed and flipped to match right scapulae. To describe scapula shape, 19 landmarks were set, as defined in Figure 1. Landmarks were digitized using TPSDig, version 1.39 (Rohlf 2006). Although the scapular blade is not entirely flat in some species, and the acromion is in a different plane than the blade, its height in the largest species, and the degree of inflexion of the borders in these species fall within the range of height differences between landmarks commonly used in analyses of 3D structures using 2D photos, and are considered negligible in this analysis (see Astúa de Moraes et al. 2000 for details). Landmarks in the coracoid and the glenoid fossa could not be included, because in lateral view the acromion partially or completely covers these structures in some of the studied species. Figure 1Open in figure viewerPowerPoint Landmarks used in this analysis, in a scapula of Lutreolina crassicaudata. The diagram represents landmarks linked for better visualization, as used in all subsequent graphs. Landmarks definition: 1, Cranial corner of the acromion-clavicle articulation; 2, Caudal corner of the acromion-clavicle articulation; 3, Caudal corner of the acromion; 4, Limit between the acromion and scapular spine; 5, Suture between the coracoid and scapular portions, when apparent, or the last apparent point of the scapular portion, when the suture is hidden by the acromion; 6, Cranial narrowing on the scapular neck; 7, Ventral end of the cranial border; 8, Cranial-most point of the cranial border; 9, Cranial angle; 10, Junction of the scapular spine with the vertebral border; 11, Dorsal-most point of the vertebral border; 12, Caudal angle; 13, Point of greater curvature (convex or concave) of the caudal border (when the caudal border was totally straight, the landmark was set equidistant from landmarks 12 and 14); 14, Caudal narrowing on the scapular neck when apparent, or the last apparent point of the caudal border, when the caudal portion of the neck is hidden by the acromion. Bar = 1 cm. I tested all landmarks for repeatability. Thirty specimens from one species were randomly selected, and all landmarks were digitized twice (on different days), randomly re-ordering the specimens between the two digitations. I estimated repeatability as the intraclass correlation coefficient (ICC), derived from an analysis of variance on the x and y coordinates of each landmark, using the individuals as factor. This takes into account the intraclass variability (error in locating landmark position) and interclass variation (real differences between individuals), following Falconer (1989). All landmarks presented repeatabilities ranging from 0.92 to 0.99 and were thus considered satisfactory and suitable for subsequent analyses. PHYLOGENY To provide a phylogenetic background against which to compare the morphological diversity in the scapula and to estimate the ancestral size and shape, a phylogenetic hypothesis for the Didelphidae generated from independent data was needed. For this purpose, I retrieved sequences of the nuclear gene IRBP from GenBank and used them to generate a phylogeny to be used in the subsequent analyses. These sequences have been produced and successfully used in a series of recent works on Didelphidae phylogeny based on morphological, karyological, and molecular data (Jansa and Voss 2000; Voss and Jansa 2003; Voss et al. 2004; Jansa and Voss 2005; Voss et al. 2005). Although other nuclear genes were available for phylogenetic reconstruction (Steiner et al. 2005; Jansa et al. 2006), the use of IRBP was preferred as it allowed the greatest number of taxa with both morphometric and genetic data (32 species, see Table 1). A neighbor-joining (NJ) tree was generated from the sequences obtained for these 32 taxa, and the resulting tree (Fig. 2) is topologically consistent with recently published estimates of didelphid phylogeny (Jansa and Voss 2000; Voss and Jansa 2003; Voss et al. 2004; Jansa and Voss 2005; Voss et al. 2005). Although Glironia has traditionally been grouped with Caluromys and Caluromysiops (e.g., Reig et al. 1987; Kirsch et al. 1997), Jansa and Voss (2000) reported slightly stronger support for the basal position of Glironia within the Didelphidae, and used it as sister group for all remaining didelphid species in subsequent analyses (e.g., Voss and Jansa 2003; Jansa et al. 2006). This tree was used to define heuristic monophyletic groups employed in the subsequent canonical variates analyses (CVAs) (see following section). Glironia and Tlacuatzin were assigned to monotypic groups to avoid clustering many other taxa into more inclusive clades that could otherwise mask the existing morphologic or taxonomic variation. Figure 2Open in figure viewerPowerPoint Phylogeny used in subsequent analyses, corresponding to a neighbor-joining unrooted tree obtained from sequences of the nuclear gene IRBP, and clades and corresponding names or abbreviations used in the labeling of figures and a priori groupings in subsequent analyses. Plot is manually rooted to keep Glironia as sister group to all other species, for clarity, but refer to text for further details on the phylogeny. Indicated nodes refer to reconstructed shapes from Table 5. GEOMETRIC MORPHOMETRICS AND MULTIVARIATE ANALYSES All landmark configurations were submitted to a Generalized Procrustes Superimposition (GPS), removing all information in the landmark coordinates related to position and orientation of the scapulae when landmarks were digitized, and to isometric size. Size is standardized by equaling centroid size (the square root of the sum of the squared distances of each landmark to the centroid of the configuration) for all landmark configurations to unit, and this size variable is used as a single measurement of size that accounts for its multivariate nature in all subsequent size analyses. This scaling removes isometric size effects only. Thus, after the GPS procedure, landmark configurations retain both shape variation unrelated to size and allometric shape variation. Further and precise details on the computations involved in geometric morphometrics can be found elsewhere (Bookstein 1991; Dryden and Mardia 1998; Zelditch et al. 2004). The existing variability in shape of the scapulae of living didelphids was assessed through a principal components analysis (PCA) of the shape variables, and the resulting PCs correspond, therefore, to the major axes of shape variation in the studied samples. Deformation grids associated with specific clustering of individuals in the PCA were then used to assess the corresponding shapes, to allow for a possible functional interpretation of this ordination. Using PCA allows detecting major patterns of shape variation and the related ordination of specimens, but PCA is not intended to separate specimens, because axes are determined on basis of the greatest variation found in the whole dataset. Therefore, shape variables were also submitted to a multivariate analysis of variance followed by a CVA, to determine if scapulae from a priori determined groups could be fully distinguished. CVAs were performed grouping individuals based on their phylogenetic relationships (to see if closely related groups of species share similarly shaped scapulae) and on their substrate use preference (to see if ecologically related species share similarly shaped scapulae). Species groupings are presented in Table 2, and corresponding clades can be visualized in Figure 2, based on a phylogenetic hypothesis obtained from an independent dataset (nuclear gene sequences, see above). Groupings into substrate use groups is based on recent reviews and studies on vertical stratification in Neotropical marsupials (Charles-Dominique et al. 1981; Grelle 2003; Vieira and Monteiro-Filho 2003; Vieira 2005a,b), as well as results from locomotor performance analyses for some of these taxa (Cunha and Vieira 2002; Delciellos and Vieira 2006). However, because weight has an allometric effect on mechanical demands on the scapulae, two sets of groupings of species were used, including or not body size (Table 2). For body size, taxa were classified as large (above 300 g, including all “big-bodied” opossums and woolly opossums), medium (approximately 100–300 g, the medium-sized bushy-tailed and gray woolly opossums), and small (below 100 g, the short-tailed and the mouse opossums). Finally, to depict the shape variation associated with each CV, shape variables were regressed onto the CV scores of each axis, and the corresponding grids for the extremes of each axis were determined. PCA was calculated with TPSRelW, version 1.45 (Rohlf 2007a), CVAs with the CVAGen module, version 6o, from the IMP (Integrated Morphometrics Package) Suite (Sheets 2004), and regressions of shape variables on CV scores with TPSRegr, version 1.31 (Rohlf 2005). Table 2. Groupings used in the labeling of the PCA and in the a priori groups in the CVAs. Refer to text for sources of data and to Figure 2 for the phylogeny used for groupings. Genus Phylogeny Substrate use Substrate use + Size Caluromys Caluromyinae Arboreal Arboreal—Large Caluromysiops Caluromyinae Arboreal Arboreal—Large Chironectes Didelphini +Metachirus Semiaquatic Semiaquatic Didelphis Didelphini +Metachirus Generalist Generalist—Large Glironia Glironia Arboreal Arboreal—Medium Gracilinanus Gracilinanus + Lestodelphys + Thylamys Arboreal Arboreal—Small Lestodelphys Gracilinanus + Lestodelphys + Thylamys Terrestrial Terrestrial—Small Lutreolina Didelphini +Metachirus Terrestrial Terrestrial—Large Marmosa Marmosa+Micoureus Scansorial Scansorial—Small Marmosops Marmosops Scansorial Scansorial—Small Metachirus Didelphini +Metachirus Terrestrial Terrestrial—Large Micoureus Marmosa+Micoureus Arboreal Arboreal—Medium Monodelphis Monodelphis Terrestrial Terrestrial—Small Philander Didelphini +Metachirus Scansorial Scansorial—Large Thylamys Gracilinanus + Lestodelphys + Thylamys Terrestrial Terrestrial—Small Tlacuatzin Tlacuatzin Scansorial Scansorial—Small Scores obtained from the PCA and the CVA analyses were regressed onto size (log (centroid size)) to estimate the influence of size in each ordination pattern. CORRELATIONS WITH ECOLOGY AND PHYLOGENY Matrix correlations were conducted to quantify the existing correlation between patterns of morphological similarity, phylogenetic relationships, and ecological similarities (reflected in preference of substrate). Matrices of morphological distances were compared with a matrix of evolutionary distance and ecological dissimilarity. These matrices were also compared to a matrix of size differences between taxa. The morphological similarity matrix was constructed using tangent plane approximations of the Procrustes distances between mean shapes for each species. Tangent distances are good estimates of the Procrustes distances, given the amount of shape variation in mammal specimens (Marcus et al. 2000), and a correlation analysis between the tangent and Procrustes distances for this dataset yielded a slope of 0.998066 and a correlation of 1. A matrix of genetic distances was used as a surrogate of phylogenetic or evolutionary distance matrix. The IRBP sequences used to construct the phylogeny were used, and pairwise distances were calculated using MEGA version 4 (Tamura et al. 2007), using the model K2P +Γ (Γ= 0.84), which showed the best fit for these sequences (Voss and Jansa 2003). Data for the ecology (substrate use) dissimilarity matrix were recovered from the literature. For each species, a value varying from 0 to 3 (0: layer never used; 1: layer rarely used; 2: layer frequently used; 3: layer used exclusively or almost exclusively) was attributed for the frequency of use of each of four substrates: floor, understory, canopy, and water. This value was converted to the proportion of use of that substrate in relation to the total use of the species, and a similarity index was calculated for each pair of species. This index varies from 0 to 1, corresponding to the sum of the values for each category for each pair, given that for each category is attributed a value corresponding to the square root of the product of the proportions of that category for each of the compared species, as proposed by Marroig and Cheverud (2001). These similarity indexes (S) were then used to compose a dissimilarity matrix, using 1 − S. Data for assigning values were obtained from the same sources used for classification of taxa into locomotor groups (see “Geometric morphometrics and multivariate analyses” above). Differences in body size may reflect differently in morphologies of taxa with similar stratum use, and thus differences in layer used only may not reflect directly in scapula morphologies. Thus, differences in locomotor habits were corrected for size, and tested again for correlation with morphology. For each pair of taxa, the ratio of the smaller centroid size to the larger one was calculated, and used as a correction factor for the similarities as calculated above. Thus, species with similar sizes (a ratio close to 1) would keep similarity indexes close to the ones calculated originally, and species with similar locomotor habits, but divergent sizes, would have their similarity diminished. As an additional test, correlations between morphology and locomotion (without correction for size), were compared using only big-bodied taxa on one side, and small- and medium-sized taxa on the other, using the same size classes as in the CVA (Table 2). For differences in size between taxa, two matrices were tested: one using the difference between log (Centroid size) of pairs of taxa and another one using ratios of centroid sizes. Both matrices performed very similarly and only results for the former are presented. All these matrices were then compared with tests of matrix correlation followed by Mantel's tests with 5000 permutations. Mantel's tests were calculated with PaSt, version 1.73 (Hammer 2007). CHARACTER RECONSTRUCTION For the reconstruction of hypothetical sizes and shapes for the scapulae at each node over the phylogeny obtained from the IRBP sequences, mean size and shape (calculated as the mean of aligned coordinates) were used as estimates for each terminal taxon (the 32 species with both genetic and morphometric data). Hypothetical sizes and shapes were then reconstructed using squared-change parsimony, using branch lengths (Maddison 1991). This parsimony criterion for reconstruction of continuous characters at ancestral nodes minimizes the sum of squared-changes on the branches, and these changes are weighed by branch lengths, so that changes on longer branches are considered less costly (Maddison 1991). Although some limitations have been shown for this method, such as big confidence intervals for the reconstructed values, it is still the best approach available to deal with continuous characters. More accurate estimates can be obtained by including fossil taxa, which were unavailable for the focus group of this study (Didelphidae), or increasing taxon sampling, which was sought here (see Finarelli and Flynn 2006 for further details and discussion). Reconstructions were conducted with TpsTree, version 1.21 (Rohlf 2007b) for scapular shapes, and with Mesquite, version 2.01 (Maddison and Maddison 2007) for scapula sizes. The NJ tree was unrooted, and as such resulted in both programs in a basal polytomy with the Caluromyinae, Glironia, and the Didelphinae. The tree was kept with this polytomy because some hypotheses group Glironia with the Caluromyinae whereas others recover it as the most basal genus in the Didelphidae (as explained above). Keeping this basal polytomy or forcing the root either between Glironia and the remaining taxa or between Didelphinae and Caluromyinae (including Glironia) did not alter significantly the estimated ancestral sizes and shapes (data not shown). Living species were used as a reference for a functional interpretation of the inferred ancestral morphologies, to facilitate interpretation of reconstructed sizes and shapes. Thus, for size estimates of some selected nodes, closest-sized species were used as a reference. For shape, all shapes (mean shapes for all actual living species—terminals—and reconstructed shapes for all nodes) were included in one single matrix, and all pairwise tangent distances were calculated with TpsSmall (Rohlf 2003). Thus, for any given node, the closest living species were used as a reference for interpretation of the reconstructed shape. Likewise, these size differences and shape distances were used to estimate the taxa with greater divergence from the overall reconstructed ancestral scapula. Results SHAPE VARIABILITY The result of the PCA on the shape variables is presented in Figure 3. Only the first three PCs contained more than 10% of the overall variation, with the first two comprising 54.10% of it (only ordination along theses two axes is presented here, as they contain the most information regarding shape). The first PC is highly correlated to size (Table 3), indicating that the shape variation along this axis is mostly allometric, with the big-bodied Didelphini, Metachirus, and most Caluromyinae toward one of its ends, contrasting with most murine and short-tailed opossums on the other, regardless of phylogenetic affinity or locomotor habits. Figure 3Open in figure viewerPowerPoint Principal components (PC) analysis of shape variables (partial warps + uniform components) of the scapulae of living species of Didelphidae, and percentage of
Referência(s)