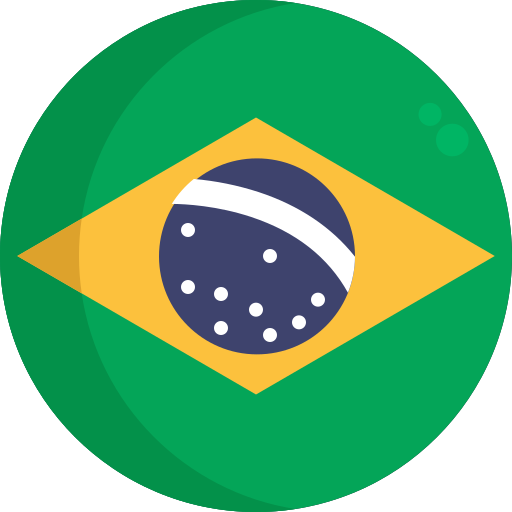
EHRA/HRS/APHRS/SOLAECE expert consensus on Atrial cardiomyopathies: Definition, characterisation, and clinical implication
2016; Elsevier BV; Volume: 32; Issue: 4 Linguagem: Inglês
10.1016/j.joa.2016.05.002
ISSN1883-2148
AutoresAndreas Goette, Jonathan M. Kalman, Luis Aguinaga, Joseph G. Akar, José Ángel Cabrera, Shih Ann Chen, Sumeet S. Chugh, Domenico Corradi, André d’Ávila, Dobromir Dobrev, Guilherme Fenelon, Mario D. González, Stéphane N. Hatem, Robert Helm, Gerhard Hindricks, Siew Yen Ho, Brian D. Hoit, José Jalife, Young‐Hoon Kim, Gregory Y.H. Lip, Changsheng Ma, Gregory M. Marcus, Katherine T. Murray, Akihiko Nogami, Prashanthan Sanders, William Uribe, David R. Van Wagoner, Stanley Nattel,
Tópico(s)Cardiac electrophysiology and arrhythmias
ResumoThe atria provide an important contribution to cardiac function [1], [2]. Besides their impact on ventricular filling, they serve as a volume reservoir, host pacemaker cells and important parts of the cardiac conduction system (e.g. sinus node, AV node), and secrete natriuretic peptides like atrial natriuretic peptide (ANP) and brain natriuretic peptide (BNP) that regulate fluid homoeostasis. Atrial myocardium is affected by many cardiac and non-cardiac conditions [3] and is, in some respects, more sensitive than ventricular [4]. The atria are activated, besides the three specialised intermodal tracts [5], [6], through working cardiomyocytes, so that any architectural or structural change in the atrial myocardium may cause significant electrophysiological disturbances. In addition, atrial cells (both cardiomyocytes and non-cardiomyocyte elements like fibroblasts, endothelial cells, and neurons) react briskly and extensively to pathological stimuli [3] and are susceptible to a range of genetic influences [7]. Responses include atrial cardiomyocyte hypertrophy and contractile dysfunction, arrhythmogenic changes in cardiomyocyte ion-channel and transporter function, atrial fibroblast proliferation, hyperinnervation, and thrombogenic changes [2]. Thus, atrial pathologies have a substantial impact on cardiac performance, arrhythmia occurrence, and stroke risk [1], [8]. Ventricular cardiomyopathies have been well classified; however, a definition and detailed analysis of ‘atrial cardiomyopathy’ is lacking from the literature. The purpose of the present consensus report, prepared by a working group with representation from the European Heart Rhythm Association (EHRA), the Heart Rhythm Society (HRS), the Asian Pacific Heart Rhythm Society (APHRS), and Sociedad Latino Americana de Estimulacion Cardiaca y Electrofisiologia (SOLAECE), was to define atrial cardiomyopathy, to review the relevant literature, and to consider the impact of atrial cardiomyopathies on arrhythmia management and stroke. The working group proposes the following working definition of atrial cardiomyopathy: ‘Any complex of structural, architectural, contractile or electrophysiological changes affecting the atria with the potential to produce clinically-relevant manifestations’ (Table 1). Many diseases (like hypertension, heart failure, diabetes, and myocarditis) or conditions (like ageing and endocrine abnormalities) are known to induce or contribute to an atrial cardiomyopathy. However, the induced changes are not necessarily disease-specific and pathological changes often share many similarities [9], [10]. The extent of pathological changes may vary over time and atrial location, causing substantial intraindividual and interindividual differences. In addition, while some pathological processes may affect the atria very selectively (e.g. atrial fibrillation-induced remodelling), most cardiomyopathies that affect the atria also involve the ventricles to a greater or lesser extent. There is no presently accepted histopathological classification of atrial pathologies. Therefore, we have proposed here a working histological/ pathopysiological classification scheme for atrial cardiomyopathies (Table 1; Fig. 1). We use the acronym EHRAS (for EHRA/HRS/ APHRS/SOLAECE), defining four classes: (I) principal cardiomyocyte changes [11]-[15]; (II) principally fibrotic changes [10], [14], [16]; (III) combined cardiomyocyte-pathology/fibrosis [9], [11], [12]; (IV) primarily non-collagen infiltration (with or without cardiomyocyte changes) [17]-[19]. This simple classification may help to convey the primary underlying pathology in various clinical conditions. The EHRAS class may vary over time and may differ at atrial sites in certain patients. Thus, this classification is purely descriptive and in contrast to other classifications (NYHA class, CCS class etc.), there is no progression in severity from EHRAS class I to EHRAS IV (Table 2). The classification may be useful to describe pathological changes in biopsies and to correlate pathologies with results obtained from imaging technologies etc. In the future, this may help to define a tailored therapeutic approach in atrial fibrillation (AF) (1-3). Histological and pathopysiological classification of atrial cardiomyopathies (EHRA/HRS/APHRS/SOLAECE): EHRAS classification. The EHRAS class may vary over time in the cause of the disease and may differ at various atrial sites. Of note, the nature of the classification is purely descriptive. EHRAS I–IV is not intended to describe disease progression from EHRAS I to EHRAS IV. (A) EHRAS Class I (biopsy): there are severe changes affecting ‘primarily’ the cardiomyocytes in terms of cell hypertrophy and myocytolysis; fibrosis is much less evident than myocyte modifications. (B) EHRAS Class II (biopsy): cardiomyocyte alterations are relatively modest compared with severe fibrotic changes; in this case, interstitial changes are much more prevalent than myocyte ones. (C) EHRAS Class III (biopsy): this is a combination of cardiomyocyte changes and collagen fibre deposition. (D) EHRAS Class IV (autopsy heart): primarily neutro-philic myocarditis. EHRAS Class IV (autopsy heart): this image shows a myocardial interstitial with some fibrosis but prominent amyloid (AL type) deposition (left-hand side, congo red staining under regular light microscope; right-hand side, congo red staining under polarised light microscope). Each atrium has a morphologically characteristic atrial body and appendage (Fig. 4). In the body, there is a venous component with the orifices of the systemic or pulmonary veins (PVs) and a vestibular component that surrounds the atrial outlet [20]. The interatrial septum (IAS) separates the atrial bodies. The venous component of the left atrium (LA) is located posterosuperiorly and receives the PVs at the four corners, forming a prominent atrial dome. The LA is situated more posteriorly and superiorly than the right atrium separated by the obliquity of the plane of the IAS [21]. Schematic representations and heart dissections to show the arrangement of the myocardial strands in the superficial parts of the walls. (A) The dissection viewed from the anterior aspect display the interatrial muscle Bachmann bundle and its bifurcating branches leftward and rightward. (B) A view of the roof and posterior wall of the left and right atriums. The right pulmonary veins (PVs) passes behind the intercaval area. The subepicardial dissection shows the abrupt changes in fibre orientation and the myocardial strands (septopulmonary bundle) in the region between the left and right PVs. The red arrows show multiple muscle bridges connecting the two atria. ICV, inferior caval vein; LAA, left atrial appendage; LSPV, left superior pulmonary vein; MV, mitral valve; RAA, right atrial appendage; RIPV, right inferior pulmonary vein; RSPV, right superior pulmonary vein; SCV, superior caval vein; TV, tricuspid valve (see text for details). The LA appendage (LAA) is smaller than the right atrium appendage (RAA). Narrower and with different shapes has a distinct opening to the atrial body and overlies the left circumflex coronary artery. Its endocardial aspect is lined by a complex network of muscular ridges and mem-branes [22], [23]. Different LAA morphologies have been described, and it appears that LAA morphology correlates with the risk of thrombogenesis [24]. Bachmann's bundle is a broad epicardial muscular band running along the anterior wall of both atria (Fig. 4). The rightward arms extend superiorly towards the sinus node and inferiorly towards the right atrioventricular groove, while the leftward arms blend with deeper myofibres to pass around the neck of the LAA and reunite posteriorly to join the circumferential vestibule of the LA. The walls of LA are non-uniform in thickness (1–15 mm) and thicker than the right atrium [25]. Atrial cardiomyocytes are geometrically complex cylinders that sometimes bifurcate at their ends where they connect with adjacent fibres via band-like ‘intercalated discs’. This contractile syncytium is organised in well-defined bands that establish non-uniform anisotropic propagation of the atrial impulse [9], [11], [26]. The only clear light-microscopic morphological difference between atrial and ventricular cardiomyocytes is in size [27]. In paraffin-embedded human specimens, the cardiomyocyte transverse diameter is ×12 mm in the LAs vs. 20–22 mm in the ventricles [11], [28]. Atrial cardiomyocytes are mainly mononucleated; a minor fraction possess two or more nuclei. The nucleus is usually centrally located, with granular and/or condensed chromatin. The nuclear shape is influenced by fibre contraction, becoming more fusiform with longitudinal cell stretch [29]. Biochemically, atrial cardiomyocytes have greater lipid content than ventricular muscle cells [30]. Atrial cardiomyocytes share many characteristics with ventricular in terms of nucleus, contractile apparatus, cytoskeleton, and organelles [27], [29], [31], [32]. Unlike ventricular cardiomyocytes, atrial cardiomyocytes do not possess an extensive T-tubule network but they do have prominent sarcoplasmic reticulum (SR) elements known as Z-tubules [33]. Therefore, the atrial sarcolemma does not protrude into the cell, and voltage-operated Ca2+ channels mainly function at the cell periphery [34]. Atrial cardiomyocytes display specific granules (100–400 nm) situated mainly in the paranuclear area adjacent to the Golgi apparatus, which contain ANP, the BNP, and related peptides [23], [24]. Atrial interstitium consists of cellular and extracellular components (see 2-5). The cellular elements include fibroblast/myofibroblasts, adipocytes, undifferentiated mesenchymal cells, and isolated inflammatory cells. The atrial wall has a significant number of medium-sized blood vessels, especially in the sub-epicardium. Mature adipose tissue is frequently found in atrial myocardium, especially the epicardium, and often permeates the layers around intramural coronary branches. The number of adipocytes is highly variable and increases with age [27]. The extracellular components consist of collagen fibres, which form most of the myocardial skeleton, proteoglycan particles, lipidic debris, spherical micro-particles, and matrix vesicles [27]. Normal histology of the left atrium and relevant pathological changes in mitral valve disease-associated atrial fibrillation. (A) Medium-power view of a normal left atrial myocardium which is composed of large bands of homogeneous cardiomyocytes. (B) In the same atrium as in (A), the Van Gieson staining show that collagen fibres (red colour) are primarily seen in the adventitial spaces of blood vessels (arrow). (C) Low-power view of a left atrium from a patient with mitral valve disease-associated atrial fibrillation. Large bands of cardiomyocytes are separated by significant amounts of pathologic fibrous tissue (arrows). (D) In the same atrium as in (C), the Van Gieson staining shows that the pathologic fibrous significantly thickens the perivascular spaces (perivascular fibrosis, arrow) and separates single or small groups of cardiomyocytes (interstitial fibrosis, arrowhead). (E) In atrial fibrillation, a variable number of cardiomyocytes undergo loss of contractile elements starting from the perinuclear area and resulting in so-called myocytolysis. These spaces may be empty (arrow) or filled with glycogen (arrowhead). (F) A higher-power view of myocytolysis with both glycogen rich (arrow) and optically empty (arrowhead) cardiomyocytes. (G) Ultrastructural view of a myolytic cardiomyocyte with significant loss of contractile elements around the nucleus (asterisk). In this empty area, there is very often accumulation of mitochondria (arrowhead) while the adjacent myofibrils display signs of abnormal contraction (arrow). (H) An LA from a patient with atrial fibrillation where the myocardial microcirculation (arrow) is slightly reduced and irregularly distributed. Stainings. (A and C) haematoxylin–eosin staining; (B and D) Van Gieson staining for collagen; (E and F) Periodic acid Schiff staining; (G) ultrastructural image; (H) immunohistochemical analysis with an anti-CD31 antibody. Original magnifications. (A, B, E, and H) ×20; (C and D) ×4; (F) ×40; (G) ×2800. Collagen fibres, mainly type I, are both normal and essential components (1-5). Atrial fibrous tissue may be sub-divided into pure interstitial and perivascular (or adventitial). Interstitial collagen fibres represent ×5% of the atrial wall volume. The atrial myocardium is also the site of sparse postganglionic nerve endings (from the ‘intrinsic cardiac nervous system’), mostly within discrete fat pads but also among cardiomyocytes [35]. The atria have a number of electrophysiological features that distinguish them from the ventricles and govern their arrhythmia susceptibility. Atrial cardiomyocytes have distinct action potential (AP) properties from ventricular cardiomyocytes, resulting in a large part from distinct ion-channel properties and distribution (Fig. 6A) [36], [37]. Atrial background inward-rectifier K+ current (IK1) is smaller than that of ventricular K+ current, resulting in a less negative resting potential and more gradual slope of phase-3 repolarization. Atrial cells also have two K+-currents that are absent in ventricle cells: the ultrarapid delayed rectifier current (IKur) and the acetylcholine-regulated K+-current (IKACh). In addition, there is evidence that atrial Na+-current has different properties compared with ventricular current [38]. As well as distinctions between atrial and ventricular APs, different atrial regions may have discrete AP and ion-channel properties [37], [39]. These cellular electrophysiological characteristics have implications for antiarrhythmic drug action and design, and may also affect the responses to atrial arrhythmias and disease [36], [37]. (A) Comparison of atrial and ventricular action potential properties and underlying ionic currents. Resting potentials (2mV) are more negative (averaging 280–285mV) in ventricular vs. atrial (270–275mV) myocytes. (B) Connexin distribution differs between atria and ventricles, with connexin-43 only expressed in ventricular cardiomyocytes (CMs) but atrial CMs having both connexin-40 and connexin-43. (C) Ralistic reconstruction of the structure of sheep atria. The right atrium (RA), left atrium (LA), pectinate muscles (PM), Bachmann's bundle (BB) and pulmonary veins (PV) are colour coded. From Ref. [43] with permission. The atria have a different pattern of cell-to-cell coupling protein (connexin) distribution compared with ventricular myocardium [36]. Whereas working ventricular cardiomyocytes express connexin-43 exclusively, atrial cardiomyocytes have significant expression of connexin-40 (Fig. 6B) [36]. Heterogeneities in connexin-40 distribution are common in paroxysmal AF and may play a pathophysiological role [40], and gene variants affecting connexin-40 sequence and/or transcription predispose to AF occurrence [41]. The atria have a very complex 3D structure (Fig. 6C) not found in the ventricles. These include interatrial connections limited to Bachmann's bundle, the septum, and the CS; pectinate muscles, the crista terminalis, and fibres surrounding the coronary sinus in the right atrium; and the PVs with complex fibre orientation around them in the LA. These structural complexities have important potential implications for atrial patho-physiology and management of atrial arrhythmias [42]. Extensive recent work has gone into the realistic mathematical reconstruction of such geometric complexities [43], and they have been incorporated into analytical approaches designed to implement patient-specific arrhythmia therapies [44]. Cable-like strands of atrial tissue like the pectinate muscles and crista terminalis are organised such that conduction within them is primarily longitudinal, with an ‘anisotropy ratio’ (longitudinal/transverse conduction velocities) as great as 10, whereas in working ventricular muscle the ratio is typically more between 2 and 4 [45]. There are autonomic ganglia on the surface of the heart that are important way-stations for cardiac autonomic control [46]. Moreover, alterations in local cardiac innervation and intracardiac autonomic reflexes play an important role in physiology and arrhythmia control. Most of the cardiac autonomic ganglia are located on the atria, and in particular in the region of the PV ostia. Thus, they are well positioned to affect atrial electrical activity in regions particularly important in AF, and their alteration by therapeutic manoeuvers like PV ablation may contribute to antiarrhythmic efficacy [42], [46], [47]. The left atrial contribution to overall cardiovascular performance is determined by unique factors. First, left atrial function critically determines left ventricular (LV) filling. Second, chamber-specific structural, electrical and ion remodelling alter left atrial function and arrhythmia susceptibility. Third, atrial function is highly relevant for the therapeutic responses of AF. Fourth, LA volume is an important biomarker that integrates the magnitude and duration of LV diastolic dysfunction. The development of sophisticated, non-invasive indices of LA size, and function might help to clinically exploit the importance of LA function in prognosis and risk stratification [1], [48]. Fibre orientation of the two thin muscular layers (the fascicles of which both originate and terminate at the atrioventricular ring) introduce a complexity that challenges functional analysis. Ultrastructurally, atrial cardiomyocytes are smaller in diameter, have fewer T-tubules, and more abundant Golgi apparatus than ventricular. In addition, rates of contraction and relaxation, conduction velocity, and anisotropy differ, as does the myosin isoform composition and the expression of ion transporters, channels, and gap junctional proteins (see relevant sections). The principal role of the LA is to modulate LV filling and cardiovascular performance by operating as a reservoir for PV return during LV systole, a conduit for PV return during early LV diastole, and as a booster pump that augments LV filling during LV diastole. There is a critical interplay between these atrial functions and ventricular systolic and diastolic performance. Thus, while LA compliance (or its inverse, stiffness), and, to a lesser extent, LA contractility and relaxation are the major determinants of reservoir function during LV systole, LV end-systolic volume and descent of the LV base during systole are important contributors. Conduit function is also governed by LA compliance and is reciprocally related to reservoir function, but because the mitral valve is open in diastole, conduit function is also closely related to LV compliance (of which relaxation is a major determinant). Atrial booster-pump function reflects the magnitude and timing of atrial contractility, but also depends on venous return (atrial preload), LV end-diastolic pressures (atrial afterload), and LV systolic reserve. Left atrium booster-pump function represents the augmented LV-filling resulting from active atrial contraction (minus retrograde blood-ejection into the PVs) and has been estimated by measurements of (i) cardiac output with and without effective atrial systole, (ii) relative LV-filling using spectral Doppler of transmitral, PV, and LA-appendage flow, (iii) LA-shortening and volumetric analysis, and (iv) tissue Doppler and deformation analysis (strain and strain-rate imaging) of the LA-body [1]. Booster-pump function can also be evaluated echocardiographically by estimating the kinetic energy and force generated by LA contraction. The relative importance of the LA contribution to LV filling and cardiac output remain controversial. A load-independent index of LA contraction based on the analysis of instantaneous relation between LA pressure and volume, analogous to LV end-systolic elastance measurements, has been used as a load-independent measure of LA pump function, validated ex vivo and in the intact dog (Fig. 7) [49]. While LA pressure–volume loops can be generated with invasive and semi-invasive means in humans [50], these methods are cumbersome, time-consuming, and difficult to apply. Measurement of myocardial strain and strain rate, which represent the magnitude and rate of myocardial deformation, assessed using either tissue Doppler velocities (tissue Doppler imaging, TDI) or by 2D echocardiographic (2D speckle-tracking or STE) techniques (Fig. 8) provide objective, non-invasive measurements of LA myocardial performance and contractility that overcome these limitations [1], [51]. Left atrial pressure–volume loop. (A) Analogue recordings of left atrial pressure and dimensions in the time domain. Vertical lines indicate time of mitral valve opening (A), end of passive atrial emptying and onset of atrial diastasis (B), atrial end-diastole (C), and atrial end-systole (D). a and v represent respective venous pressure waves. (B) Left atrial pressure–volume loop from a single beat illustrating characteristic figure-of-eight configuration. Arrows indicate the direction of loop as a function of time. A loop represents active atrial contraction. V loop represents passive filling and emptying of the LA. MVO, time of mitral valve opening; MVC, approximate time of mitral valve closure; LA, left atrial end-systole; and LAd, left atrial end-diastole. Reproduced from Ref. [49] with permission. LA functions colour-coded displays of atrial functions (red, reservoir; blue, conduit; yellow, booster pump) related to events in the cardiac cycle. Displayed are pulmonary venous (PV) velocity, LA strain, LA strain rate, LA volume and pressure, and mitral spectral and tissue Doppler. Reproduced from Ref. [1] with permission. Nearly half of the LV stroke volume and its associated energy are stored in the LA during LV systole. This energy is subsequently expended during the LV diastole. Reservoir function is governed largely by atrial compliance during ventricular systole, which is measured most rigorously by fitting atrial pressures and dimensions, taken either at the time of mitral valve opening/closure over a range of atrial pressures and volumes or during ventricular diastole, to an exponential equation [52]. Although this method requires atrial dimensions and pressures, the relative reservoir function can be estimated simply with PV Doppler: the proportion of LA inflow during ventricular systole provides an index of the reservoir capacity of the atrium. Reservoir function can also be estimated from LA time–volume relations as either the total ejection fraction or distensibility fraction, calculated as the maximum minus minimum LA volume, normalised to maximal or minimal LA volume, respectively. Although largely neglected, the LA–appendage is more compliant than the LA–body [52], so the contribution of the appendage to overall LA compliance is substantial with potential negative implications for routine atrial appendectomy/ligation during mitral valve surgery. Left atrium strain and strain rates during LV systole predict successful sinus rhythm restoration following DC cardioversion or AF ablation, and are surrogates of atrial fibrosis and structural remodelling; coupled with an estimate of atrial pressure (e.g. transmitral E/E′), strain has the potential to estimate atrial distensibility non-invasively [1], [53]. Left atrium conduit function occurs primarily during ventricular diastole and represents the trasport of blood volume that cannot be attributed to either reservoir or booster-pump functions, accounting for approximately one-third of atrial flow [54]. A reciprocal relation exists between LA conduit and reservoir functions; a redistribution between these functions is an important compensatory mechanism that facilitates LV filling with myocardial ischaemia, hypertensive heart disease, and mitral stenosis (MS). Conduit function is estimated by the early diastolic transmitral flow, diastolic PV-flow, and LA strain and strain rate during early diastole. There are major differences in the expression and function of Ca2+-handling proteins between atria and ventricles (Fig. 9) [55]. The atria have reduced cardiomyocyte contraction and relaxation times and shorter Ca2+-transient duration [56]-[58]. In atria, protein levels [57], [59] and activity [57], [59] of the SR Ca2+-ATPase2a (Serca2a) are two-fold higher, whereas the Serca2a-inhibitor phospholamban (PLB) is less abundant, vs. ventricles [57], [59]. Atrial, but not ventricular, Serca2a is also regulated by sarcolipin (SLN) and SLN ablation increases atrial SR Ca2+-uptake and contractility [60]. L-type Ca2+-current [61] is similar in both chambers, whereas protein levels of ryanodine receptor type-2, calsequestrin, triadin, junction and Na2+ –Ca2+ exchanger are lower in atria than in ventricles [59], [62], [63]. In contrast to ventricular myocardium, T-tubules are less abundant in atrial cardiomyocytes [64]. In addition, atrial cardiomyocytes possess much more Ca2+-buffering mitochondria than ventricular cardiomyocytes [56]. As a consequence, the atrial Ca2+ wave starts in the myocyte periphery and then propagates to the centre of the myocyte, activating additional Ca2+-releasing sites in the SR [55]. Excitation–contraction coupling in atria vs. ventricles. Schematic representation of the cell structure and major Ca2+ handling proteins, along with related currents and ion transporters (A). Illustration of action potential (top), Ca2+ transient (middle) and confocal linescan image of intracellular Ca2+ wave propagation towards cell centre (bottom) in a ventricular (left) vs. atrial (right) cardiomyocyte (B). Arrows indicate differences in expression and/or function of Ca2+ handling proteins in atrial vs. ventricular cardiomyocytes. INa, Na+ current; FKPB12.6, FK506-binding protein 12.6; JPH2, Junctophilin-2; MyBP-CMyosin bindig protein C; TnI, Troponin-I; for further abbreviations, see text. ‘Lone’ atrial fibrillation (LAF) is diagnosed when no apparent explanation or underlying comorbidity can be identified [65], [66]. Over the last few years, new epidemiological associations with AF have emerged and the number of true LAF cases has progressively decreased [67]. Like AF associated with comorbidities, LAF occurs more frequently in males than in females with a ratio of 3–4:1 [68]. Recent studies have shown that true cases of LAF can be diagnosed even in subjects older than 60 years, so that this age limit seems inappropriately conservative [69]. At the same time, it is unclear whether cases with left atrial enlargement should be excluded from the LAF category. In fact, LA enlargement might even be the consequence of the arrhythmia [70]. ‘Lone’ atrial fibrillation is at the lower end of the thromboembolic risk spectrum, with only a 1–2% cumulative 15-year risk of stroke [66]. How-ever, with ageing and/or the occurrence of cardiovascular comorbidities, the risk of AF-related complications (including thromboembolic events) increases [71]. Patients originally diagnosed with LAF may follow different clinical courses based on their left atrial volume: individuals who retain normal LA size throughout long-term follow-up show a relatively benign course, while those with LA enlargement experience adverse events like stroke, myocardial infarction, and heart failure [72]. The majority of LAF patients first present with paroxysmal episodes and show low progression rates into permanent AF [71], [73]. Atrial fibrillation has clear genetic determinants [7]. These include common gene variants with low predictive strength and rare gene mutations that have much greater penetrance [7]. Frustaci et al [14]. explored the histological morphology of right atrial septal biopsies from patients with lone paroxysmal AF, finding chronic inflammatory infiltrates, foci of myocyte necrosis, focal replacement fibrosis, and myocyte cytoplasmic vacuoles consistent with myolysis. Of their 12 patients, 10 showed EHRAS class III changes and 2 showed EHRAS class II. Stiles et al [74]. found bi-atrial structural change, conduction abnormalities, and sinus node dysfunction in paroxysmal LAF patients. Skalidis et al [75]. demonstrated atrial perfusion abnormalities and coronary flow reserve impairment. Much more recently, morphometric assessment of atrial biopsies from the LA posterior wall of persistent or long-lasting persistent LAF patients demonstrated cardiomyocyte hypertrophy, myolytic damage, interstitial fibrosis, and reduced connexin-43 expression vs. controls [76]. The accumulation of insoluble, misfolded proteins is linked to an increasing number of age-related degenerative diseases [77]. Amyloidosis represent the deposition of insoluble, fibrillar proteins in a cross b-sheet structure that characteristically binds dyes such as Congo red. The most common form of age-related or senile amyloidosis is limited to the atrium, a condition known as isolated atrial amyloidosis (IAA) [17], [78]. The incidence of atrial amyloidosis increases with age, exceeding 90% in the ninth decade [79]. Isolated atrial amyloidosis is also linked to structural heart disease. In atrial biopsies from 167 patients undergoing cardiac surgery, 23 of 26 amyloid-positive specimens were from patients with rheumatic heart disease (RHD), while the remaining 3 came from patients with atrial septal defects [80]. The overall incidence of 16% was greater than that was seen in control atrial autopsy specimens from trauma victims (3%). Histologically, IAA is classified as EHRAS IVa (Fig. 3; Table 2). Atrial natriuretic peptide is a fibrillogenic protein that forms IAA [81]. Amyloid deposits are immunoreactive for ANP in most patients [17], while transthyretin, a transport protein implicated in systemic senile amyloidosis, was also identified in 10% [4] (NT-pro-ANP has been identified in
Referência(s)